Abstract
The neuron-specific transcription factor T-box brain 1 (TBR1) regulates brain development. Disruptive mutations in the TBR1 gene have been repeatedly identified in patients with autism spectrum disorders (ASDs). Here, we show that Tbr1 haploinsufficiency results in defective axonal projections of amygdalar neurons and the impairment of social interaction, ultrasonic vocalization, associative memory and cognitive flexibility in mice. Loss of a copy of the Tbr1 gene altered the expression of Ntng1, Cntn2 and Cdh8 and reduced both inter- and intra-amygdalar connections. These developmental defects likely impair neuronal activation upon behavioral stimulation, which is indicated by fewer c-FOS–positive neurons and lack of GRIN2B induction in Tbr1+/− amygdalae. We also show that upregulation of amygdalar neuronal activity by local infusion of a partial NMDA receptor agonist, d-cycloserine, ameliorates the behavioral defects of Tbr1+/− mice. Our study suggests that TBR1 is important in the regulation of amygdalar axonal connections and cognition.
This is a preview of subscription content, access via your institution
Access options
Access to this article via Institution of Civil Engineers Library is not available.
Subscribe to this journal
Receive 12 print issues and online access
$209.00 per year
only $17.42 per issue
Buy this article
- Purchase on SpringerLink
- Instant access to full article PDF
Prices may be subject to local taxes which are calculated during checkout
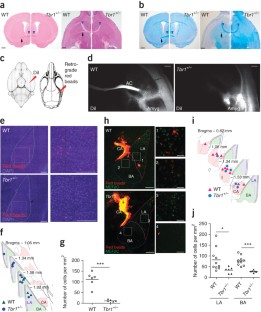
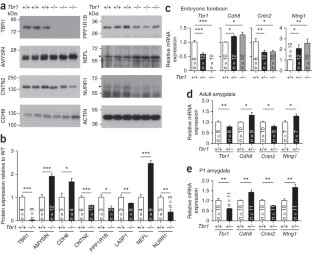
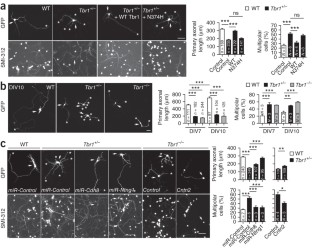
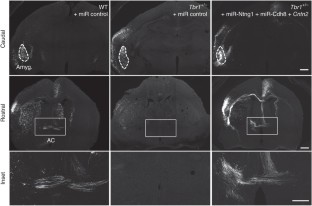
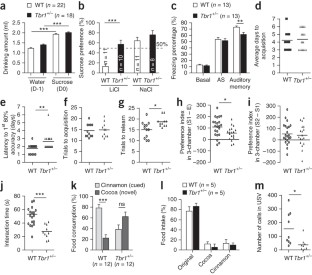
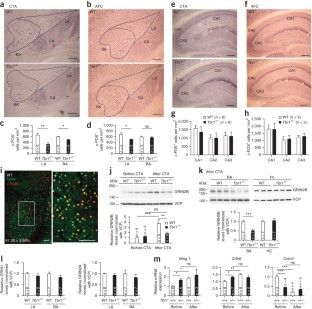
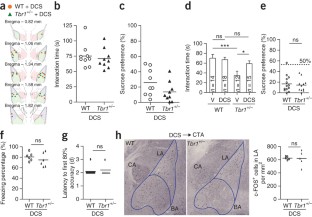
Similar content being viewed by others
Accession codes
References
Bulfone, A. et al. T-brain-1: a homolog of Brachyury whose expression defines molecularly distinct domains within the cerebral cortex. Neuron 15, 63–78 (1995).
Remedios, R. et al. A stream of cells migrating from the caudal telencephalon reveals a link between the amygdala and neocortex. Nat. Neurosci. 10, 1141–1150 (2007).
Hevner, R.F. et al. Tbr1 regulates differentiation of the preplate and layer 6. Neuron 29, 353–366 (2001).
Yu, T.W. et al. Using whole-exome sequencing to identify inherited causes of autism. Neuron 77, 259–273 (2013).
Iossifov, I. et al. De novo gene disruptions in children on the autistic spectrum. Neuron 74, 285–299 (2012).
O'Roak, B.J. et al. Sporadic autism exomes reveal a highly interconnected protein network of de novo mutations. Nature 485, 246–250 (2012).
Neale, B.M. et al. Patterns and rates of exonic de novo mutations in autism spectrum disorders. Nature 485, 242–245 (2012).
Sanders, S.J. et al. De novo mutations revealed by whole-exome sequencing are strongly associated with autism. Nature 485, 237–241 (2012).
O'Roak, B.J. et al. Exome sequencing in sporadic autism spectrum disorders identifies severe de novo mutations. Nat. Genet. 43, 585–589 (2011).
O'Roak, B.J. et al. Multiplex targeted sequencing identifies recurrently mutated genes in autism spectrum disorders. Science 338, 1619–1622 (2012).
LeDoux, J. The amygdala. Curr. Biol. 17, R868–R874 (2007).
Hsueh, Y.P., Wang, T.F., Yang, F.C. & Sheng, M. Nuclear translocation and transcription regulation by the membrane-associated guanylate kinase CASK/LIN-2. Nature 404, 298–302 (2000).
Wang, G.S. et al. Transcriptional modification by a CASK-interacting nucleosome assembly protein. Neuron 42, 113–128 (2004).
Wang, T.F. et al. Identification of Tbr-1/CASK complex target genes in neurons. J. Neurochem. 91, 1483–1492 (2004).
Huang, T.N. & Hsueh, Y.P. CASK point mutation regulates protein-protein interactions and NR2b promoter activity. Biochem. Biophys. Res. Commun. 382, 219–222 (2009).
Furley, A.J. et al. The axonal glycoprotein TAG-1 is an immunoglobulin superfamily member with neurite outgrowth-promoting activity. Cell 61, 157–170 (1990).
Stoeckli, E.T. & Landmesser, L.T. Axonin-1, Nr-CAM, and Ng-CAM play different roles in the in vivo guidance of chick commissural neurons. Neuron 14, 1165–1179 (1995).
Kunz, S. et al. Neurite fasciculation mediated by complexes of axonin-1 and Ng cell adhesion molecule. J. Cell Biol. 143, 1673–1690 (1998).
Nakashiba, T., Nishimura, S., Ikeda, T. & Itohara, S. Complementary expression and neurite outgrowth activity of netrin-G subfamily members. Mech. Dev. 111, 47–60 (2002).
Bekirov, I.H., Nagy, V., Svoronos, A., Huntley, G.W. & Benson, D.L. Cadherin-8 and N-cadherin differentially regulate pre- and postsynaptic development of the hippocampal mossy fiber pathway. Hippocampus 18, 349–363 (2008).
Muller, C.W. & Herrmann, B.G. Crystallographic structure of the T domain-DNA complex of the Brachyury transcription factor. Nature 389, 884–888 (1997).
Won, H. et al. Autistic-like social behaviour in Shank2-mutant mice improved by restoring NMDA receptor function. Nature 486, 261–265 (2012).
Horio, M., Mori, H. & Hashimoto, K. Is D-cycloserine a prodrug for D-serine in the brain? Biol. Psychiatry 73, e33–e34 (2013).
Bauer, E.P., Schafe, G.E. & LeDoux, J.E. NMDA receptors and L-type voltage-gated calcium channels contribute to long-term potentiation and different components of fear memory formation in the lateral amygdala. J. Neurosci. 22, 5239–5249 (2002).
Holmes, N.M., Parkes, S.L., Killcross, A.S. & Westbrook, R.F. The basolateral amygdala is critical for learning about neutral stimuli in the presence of danger, and the perirhinal cortex is critical in the absence of danger. J. Neurosci. 33, 13112–13125 (2013).
Hong, C.J. & Hsueh, Y.P. Cytoplasmic distribution of T-box transcription factor Tbr-1 in adult rodent brain. J. Chem. Neuroanat. 33, 124–130 (2007).
Liu, H.-Y. et al. TLR7 negatively regulates dendrite outgrowth through the Myd88-c-Fos-IL-6 pathway. J. Neurosci. (in the press) (2013).
Peter, M. et al. Transgenic mouse models enabling photolabeling of individual neurons in vivo. PLoS ONE 8, e62132 (2013).
Huang, T.N., Chang, H.P. & Hsueh, Y.P. CASK phosphorylation by PKA regulates the protein-protein interactions of CASK and expression of the NMDAR2b gene. J. Neurochem. 112, 1562–1573 (2010).
Ruel, J. et al. Salicylate enables cochlear arachidonic-acid-sensitive NMDA receptor responses. J. Neurosci. 28, 7313–7323 (2008).
Wang, H.F. et al. Valosin-containing protein and neurofibromin interact to regulate dendritic spine density. J. Clin. Invest. 121, 4820–4837 (2011).
Lin, C.W., Liu, H.Y., Chen, C.Y. & Hsueh, Y.P. Neuronally-expressed Sarm1 regulates expression of inflammatory and antiviral cytokines in brains. Innate Immun. 20, 161–172(2014).
Brose, N. et al. Differential assembly of coexpressed glutamate receptor subunits in neurons of rat cerebral cortex. J. Biol. Chem. 269, 16780–16784 (1994).
Carloni, M. et al. The impact of early life permethrin exposure on development of neurodegeneration in adulthood. Exp. Gerontol. 47, 60–66 (2012).
Poliak, S. et al. Juxtaparanodal clustering of Shaker-like K+ channels in myelinated axons depends on Caspr2 and TAG-1. J. Cell Biol. 162, 1149–1160 (2003).
Yokoyama, K. et al. NYAP: a phosphoprotein family that links PI3K to WAVE1 signalling in neurons. EMBO J. 30, 4739–4754 (2011).
Chen, C.Y., Lin, C.W., Chang, C.Y., Jiang, S.T. & Hsueh, Y.P. Sarm1, a negative regulator of innate immunity, interacts with syndecan-2 and regulates neuronal morphology. J. Cell Biol. 193, 769–784 (2011).
Sun, S.W., Song, S.K., Hong, C.Y., Chu, W.C. & Chang, C. Improving relative anisotropy measurement using directional correlation of diffusion tensors. Magn. Reson. Med. 46, 1088–1092 (2001).
Lin, C.-W. & Hsueh, Y.-P. Sarm1, a neuronal inflammatory regulator, controls social interaction, associative memory and cognitive flexibility in mice. Brain Behav. Immun. (in the press) (2013).
Chung, W.C., Huang, T.N. & Hsueh, Y.P. Targeted deletion of CASK-interacting nucleosome assembly protein causes higher locomotor and exploratory activities. Neurosignals 19, 128–141 (2011).
Hsueh, Y.P. et al. Direct interaction of CASK/LIN-2 and syndecan heparan sulfate proteoglycan and their overlapping distribution in neuronal synapses. J. Cell Biol. 142, 139–151 (1998).
Lesscher, H.M. et al. Amygdala protein kinase C epsilon regulates corticotropin-releasing factor and anxiety-like behavior. Genes Brain Behav. 7, 323–333 (2008).
Moy, S.S. et al. Mouse behavioral tasks relevant to autism: phenotypes of 10 inbred strains. Behav. Brain Res. 176, 4–20 (2007).
Colacicco, G., Welzl, H., Lipp, H.P. & Wurbel, H. Attentional set-shifting in mice: modification of a rat paradigm, and evidence for strain-dependent variation. Behav. Brain Res. 132, 95–102 (2002).
Birrell, J.M. & Brown, V.J. Medial frontal cortex mediates perceptual attentional set shifting in the rat. J. Neurosci. 20, 4320–4324 (2000).
Garner, J.P., Thogerson, C.M., Wurbel, H., Murray, J.D. & Mench, J.A. Animal neuropsychology: validation of the Intra-Dimensional Extra-Dimensional set shifting task for mice. Behav. Brain Res. 173, 53–61 (2006).
Tabata, H. & Nakajima, K. Labeling embryonic mouse central nervous system cells by in utero electroporation. Dev. Growth Differ. 50, 507–511 (2008).
Matsuda, T. & Cepko, C.L. Electroporation and RNA interference in the rodent retina in vivo and in vitro. Proc. Natl. Acad. Sci. USA 101, 16–22 (2004).
Wang, W.Y. et al. Interaction of FUS and HDAC1 regulates DNA damage response and repair in neurons. Nat. Neurosci. 16, 1383–1391 (2013).
Sananbenesi, F. et al. A hippocampal Cdk5 pathway regulates extinction of contextual fear. Nat. Neurosci. 10, 1012–1019 (2007).
Acknowledgements
We thank R. Hevner (University of Washington, Seattle) for the Tbr1+/− mice; the Functional and Micro-Magnetic Resonance Imaging Center (supported by the National Research Program for Genomic Medicine, National Science Council, Taiwan, NSC99-3112-B-001-020); S.-Y. Tung, the MicroArray Facility and the Bioinformatics Core of the Institute of Molecular Biology and DNA MicroArray Core Facility of the Institute of Plant and Microbial Biology, Academia Sinica; J. Kung and Animal Facility of Institute of Molecular Biology, Academia Sinica; C.-Y. Chang, W.-R. Wong and C.-W. Tsai for technical assistance; C. Cepko for pCAG-GFP; and M. Loney for English editing. This work was supported by Academia Sinica and the National Science Council of Taiwan (NSC 102-2321-B-001-054 and 102-2321-B-001-029 to Y.-P. H.). T.-N. H. was supported by National Science Council (NSC 102-2811-B-001-060). C.-Y. C. was also supported by National Science Council (NSC 102-2811-B-001-037).
Author information
Authors and Affiliations
Contributions
T.-N.H., H.-C.C. and Y.-P.H. contributed to the study design and analyses of data and drafted the manuscript. T.-N.H. performed the ultrasonic vocalization, novel object recognition, histological analyses, DiI tracing, retrograde labeling, local infusion and following behavioral analyses, sequences and microarray analyses. H.-C.C. performed the studies using cultured amygdalar neurons, IUE, quantitative PCR, the rest of behavioral assays and gene expression analyses after behavioral stimulation. S.-J.C. contributed to Supplementary Figure 2c–e, technical support on IUE and discussion. W.-H.C. performed the microarray analysis. C.-Y.C. and H.-F.W. confirmed the TBR1 target genes using immunoblotting. T.-N.H. and H.-C.C. contributed equally to this study.
Corresponding author
Ethics declarations
Competing interests
The authors declare no competing financial interests.
Integrated supplementary information
Supplementary Figure 1 The anterior commissure is smaller in Tbr1+/− mice.
(a) The TBR1 protein levels in Tbr1−/−, Tbr1+/− and wild-type (WT) mice at embryonic day 18.5 (E18.5). TUBULIN and VCP were used as internal controls. Total three mice for each genetic background had been examined. Only the data of two mice are shown. (b) The representative images of Nissl stain of E17.5 mouse brains. Total 4 WT, 6 Tbr1+/− and 4 Tbr1−/− brains were examined. The results were the same with the images shown here. (c) Immunostaining with CUX1, a marker of cortical projection neurons in layers 2–3, at E17.5. Similar results were found in all of three animals examined for each group. Both (b) and (c) indicate defective lamination of the cerebral cortex in Tbr1−/− mice, but not Tbr1+/− mice. (d) Magnetic resonance images (MRI) of Tbr1+/− and wild-type brains. The brain images represent a series of scans (caudal to rostral) in WT and Tbr1+/− mice. The red arrowheads point to the position of the anterior commissures (ACs). (e)-(g) Quantitative analyses of the MRI images. (e) The size of the whole brain. Each dot indicates the results obtained from an individual animal. The horizontal lines represent the means. (f) The size of the ventricles, including the 3rd, 4th, and lateral (LV) ventricles and the cerebral aqueduct (Aq). (g) Fractional anisotropy (FA, right panel) and relative anisotropy (RA, left panel) demonstrated similar sizes in the corpus callosum (CC). AC was smaller in Tbr1+/− mice. The data are presented as the mean plus s.e.m. (N = 6). ***P < 0.001. Scale bars: (b) 500 μm; (c) 50 μm.
Supplementary Figure 2 Area patterning and layer formation are not affected in Tbr1+/− mice.
(a) The size of the barrel cortex in the Tbr1+/− mouse is comparable to wild-type littermates. At postnatal day 21, cytochrome oxidase staining was performed to label the mitochondria, which were specifically condensed at the thalamic axonal termini innervating the layer IV of the barrel cortex. (b) Quantification of the barrel sizes; B1-B5, C1-C7 and D1-D7. Tbr1+/− mice and wild-type littermates showed no significant differences in barrel cortex size. (c) Serotonin immunostaining of tangential sections in layer 4 of P7 wild-type (top) and Tbr1+/− (bottom) cortices. Rostral is to the left and medial is at the top. Serotonin staining revealed the primary sensory areas, including the primary somatosensory (S1) and visual (V1) areas. (d) The total area of the cortex (Ctx), ratio of the length of the neocortex (TL), length of the caudal cortex (CL, the length from the C1 barrel to the caudal end of the cortex) and ratio of the cortical total area and V1 area size were similar in wild-type and Tbr1+/− mice. (e) In situ hybridization was performed on serial sagittal sections of P7 wild-type and Tbr1+/− cortices (rostral to left, dorsal to top). The expression of the area- and layer-specific markers showed no differences between the wild-type and Tbr1+/− brains in area patterning and layer formation. The experiments were repeated in 2 wild-type and 3 Tbr1+/− mice. *Note, Cdh8 and Rorb were also identified as TBR1 target genes in the microarray analyses. The results of the in situ hybridization also indicated an upregulation in Cdh8 and downregulation in Rorβ in the Tbr1+/− brains, which is consistent with the microarray data (Supplementary Table 1). H, hippocampus. Scale bars: (a) 500 μm; (c) 250 μm; (e) 200 μm.
Supplementary Figure 3 Connections from the sensory and motor cortex to the thalamus are normal in the Tbr1+/− mouse.
DiI crystals were implanted in the (a) sensory cortex and (b) motor cortex. Eight weeks later, the brains were either coronally (a) or sagitally (b) sectioned. (a) The cortico-cortical and corticothalamic projections in the sensory cortex were still preserved in the Tbr1+/− mice. (b) The corticothalamic connections in the primary motor cortex indicated that the motor cortical projections were present in the Tbr1+/− mice. The experiments were repeated in six animals for each group. CC, corpus callosum; CP, cerebellar peduncle; IC, internal capsule; LF, lenticular fasciculus; Th, thalamus. Scale bars: 500 μm.
Supplementary Figure 4 TBR1 is expressed in the projection neurons, but not in the inhibitory interneurons in the amygdala.
Double immunofluorescence staining with TBR1 and CaMKII (upper panel) and GAD67 (lower panel) was performed. (a) Lateral amygdala. (b) Basal amygdala. Higher magnification images of the insets are shown in the far right panels. The experiments were repeated using three WT mice. Scale bars: 20 μm.
Supplementary Figure 5 The size and identity of the lateral and basal amygdala show no obvious changes in the Tbr1+/− mice.
(a) The expression of TBR1 in the lateral (LA) and basal amygdala (BA) in the adult mouse brain was examined by fluorescent immunostaining. The experiments were repeated at least three times using different animals. (b)-(c) A DAB stain using MEF2C and ER81 antibodies. MEF2C is a marker of the lateral amygdala. ER81 is a marker of the basal amygdala. Similar results were collected from three mice for each group. (d) Acetylcholinesterase staining sharply labeled the nucleus of the amygdala. (e) Quantification of the sizes of the lateral and basal nuclei of the amygdala according to the acetylcholinesterase staining. (f) A DAPI stain revealed the cell number in the lateral (outlined in red) and basal amygdala (outlined in green). (g) Quantification of the cell density in the lateral and basal amygdala. The cell densities are similar in the Tbr1+/− and WT adult mice. The data are presented as the means plus s.e.m.. The sample size (n) indicates the number of animals in each group. Scale bars: (a) 200 μm; (b)-(d) 500 μm; (f) 200 μm.
Supplementary Figure 6 In utero electroporation of wild-type and Tbr1+/− embryos.
In utero electroporation was performed at E12.5-13.5 under conditions identical to those outlined in Figure 4. (a) An illustration of the relative position of electrodes and embryo. TBR1-positive amygdaloid nuclei originate in a unique domain at the caudal telencephalic pole. During the developmental process, they migrate rostrally from their caudal origin. To deliver plasmid DNAs into the amygdala in the embryonic brain, the positive electrode was placed close to the ventrocaudal part of fetal forebrains as shown in figure. The plasmids had a much lower possibility of labeling the rostral part of the forebrain, particularly the olfactory bulb, which extends the axons to the anterior part of AC. Thus this condition is able to label the posterior part but not anterior part of the anterior commissure. C, caudal; D, dorsal; R, rostral; V, ventral. (b) Two examples of embryos that were labeled strongly by GFP at the cerebral cortex but not the amygdala are shown. One of them is a wild-type (WT) embryo transfected with control vector; the other is Tbr1+/− embryo transfected with miR-Ntng1, miR-Cdh8 and Cntn2. Anterior commissures in these embryos could not be labeled by GFP. For wild-type mice, at least ten embryos had no GFP signal at amygdala. For Tbr1+/− mice transfected with miR-Ntng1, miR-Cdh8 and Cntn2, at least 6 embryos had no GFP signal at amygdala. None of these embryos had GFP-positive signals at the anterior commissure. Thus, the GFP-positive axons in the posterior part of AC shown in Figure 4 were likely extended from the amygdala. (c) Neuronal morphology of amygdalar, cortical and striatal neurons of IUE embryos. The same brain sections examined in Figure 4 were analyzed under high magnification. The Z-series confocal images were projected to view the entire morphology of cells. The thickness in mm of Z-series is indicated in each image. Scale bars: (b) caudal and rostral, 500 μm; inset, 200 μm; (c) 20 μm.
Supplementary Figure 7 Behavioral analyses of wild-type and Tbr1+/− mice.
(a)-(d) Tbr1+/− mice behave normally in open field, elevated plus maze, contextual fear conditioning and novel object recognition. (a) Open field test. Several parameters were measured, including the total distance traveled, speed, degree of anxiety and the total number of rearing and grooming activities. (b) Elevated plus maze test. The percentages of time that the animals spent in the open arms, closed arms, and central regions of the maze, are indicated. (c) Contextual fear conditioning. The freezing responses of Tbr1+/− mice were comparable to those of WT littermates. (d) Novel object recognition is also normal in Tbr1+/− mice. (e)-(g) Bilateral infusion of ifenprodil into the BLA of WT mice impairs social interaction and associative memory. (e) Infusion sites. (f) Reciprocal social interaction. (g) Sucrose preference in CTA. (h) A simple flowchart of CTA. (a), (d), (f), (g) Each dot indicates the results obtained from an individual animal. The horizontal lines represent the means. (b)-(c) Data are presented as the means plus s.e.m.. Sample size (n) indicates the number of animals in each group. ***P < 0.001.
Supplementary Figure 8 D-cycloserine does not upregulate the protein levels of TBR1 target genes in the amygdala.
Mice were intraperitoneally injected with D-cycloserine (20 mg kg-1) thirty min before behavioral analysis. After behavioral stimulation, lateral amygdalae were collected for immunoblotting with various antibodies as indicated. (a)-(b) The results of GRIN2B expression after CTA. Each dot indicates the results obtained from an individual animal. The horizontal lines represent the means. (c)-(d) The expressions of GRIN2B, NTNG1, CDH8, CNTN2, PPP1R1B, AMYSIN, LASP1, NEFL and NURR1 in WT mice after AFC. VCP was used as an internal control. Data are presented as the means ± s.e.m.. *P < 0.05.
Supplementary Figure 9 Full blots for all experiments.
(a) Figure 2a. (b) Figure 6j. (c) Figure 6k. (d) Supplementary figure 1a. (e) Supplementary figure 8a. (f) Supplementary figure 8c. Arrowed lines indicate the regions used in the figures.
Supplementary information
Supplementary Text and Figures
Supplementary Figures 1–9 and Supplementary Table 1 (PDF 24831 kb)
Rights and permissions
About this article
Cite this article
Huang, TN., Chuang, HC., Chou, WH. et al. Tbr1 haploinsufficiency impairs amygdalar axonal projections and results in cognitive abnormality. Nat Neurosci 17, 240–247 (2014). https://doi.org/10.1038/nn.3626
Received:
Accepted:
Published:
Issue Date:
DOI: https://doi.org/10.1038/nn.3626